Research progress of intestinal microbiota in targeted therapy and immunotherapy of colorectal cancer
Abstract
Colorectal cancer (CRC) is one of the most common malignancies of the digestive tract, with increasing morbidity and mortality worldwide, and is the third most common malignancy in the world. At present, the main treatment methods for CRC include surgery, chemotherapy, targeted therapy, and immunotherapy. Regulation of the gut microbiota is one of the most promising new strategies for CRC treatment. Gut microbiota interacts with host cells to regulate many physiological processes, such as energy acquisition, metabolism, and immune responses. Recent studies have found that a combination of gut microbiota with targeted therapy and immunotherapy could improve the therapeutic effect of colon cancer compared with treatment alone. This article reviews the mechanism of microbiota regulation in CRC and the latest progress of intestinal microbiota in targeted therapy and immunotherapy of CRC, which is helpful in developing potential prevention or treatment strategies for colorectal cancer.
Keywords
INTRODUCTION
Colorectal cancer (CRC) is the second leading cause of cancer-related death worldwide, with a 5-year survival rate of 15%[1]. With the deepening of research, more and more factors increasing the incidence of colorectal cancer have been discovered, such as cancers of unknown primary (CUP), metastatic cancer with no identifiable primary site following adequate evaluation[2]. Early screening has been shown to improve CRC survival in developed countries. While surgery alone or combined with radiotherapy or chemotherapy has been the mainstay of CRC treatment, targeted therapies such as cetuximab (Cet) and bevacizumab (Bev) have led to significantly improved overall survival since their development in the 21st century[3]. Even though older patients with pT4 disease are more prone to severe postoperative complications, there is no consensus that age affects survival outcomes. The prognosis of older patients may be confounded by differences in stage at presentation, tumor site, preexisting comorbidities, and type of treatment received[4]. There are two main chemotherapy regimens for the treatment of colorectal cancer: FOLFOX [5-fluorouracil (5-FU), leucovorin, and oxaliplatin] and FOLFIRI (5-FU, leucovorin, and irinotecan). Cet, panitumumab (Pan), and Bev are often added to these two chemotherapy regimens for synergistic therapeutic effects[5]. In recent years, immunotherapy has achieved great therapeutic effects in solid tumors such as melanoma and lung cancer; this success has led to its gradual application in treating CRC[6]. Microsatellite instability (MSI) is a change in the number of short repetitive sequences (1-6 bases) in DNA and is a form of genetic instability in tumors. dMMR is a system for correcting errors during DNA replication, and if MMR is defective, it can lead to MSI. In general, CRC patients with high MSI (MSI-H) or MMR deficiency (dMMR) have better outcomes for ICI, while CRC patients with low MSI (MSI-L) or microsatellite stabilization (MSS) have ineffective or limited treatment for ICI.
The recommended screening for defective DNA mismatch repair includes immunohistochemistry (IHC) and/or MSI test. However, there are challenges in distilling the biological and technical heterogeneity of MSI testing down to usable data. It has been reported in the literature that IHC testing of the mismatch repair machinery may give different results for a given germline mutation, and it has been suggested that this may be due to somatic mutations[7]. However, prognosis remains poor for many CRC patients[8], highlighting the need for more effective treatments.
The intestinal microbiota represents an integral part of the gastrointestinal microenvironment and has been implicated in CRC development and progression, as well as response to therapy. Under homeostatic conditions, commensal microbes confer resistance against pathogens in part by regulating epithelial cell proliferation and DNA damage[9]. Thus, maintaining the gut microbial ecosystem appears critical for CRC prevention and treatment. There is mounting evidence that the intestinal microbiota contributes to CRC via various mechanisms. Consequently, preservation of gut microbial balance could mitigate CRC advancement[10]. Furthermore, modulation of the microbiota may improve outcomes for immunotherapy and targeted therapy.
MICROBIOTA IN TARGETED THERAPY
Fusobacterium nucleatum
Fusobacterium nucleatum (F. nucleatum) is a gram-negative, spore-free anaerobic bacterium. It mainly colonizes the mouth and is an opportunistic pathogen that may cause oropharyngeal and external oral diseases[11]. In recent years, a large number of tissue samples and stool samples of clinical patients with colorectal cancer have been detected with an increased abundance of F. nucleatum[12]. Studies have shown that F. nucleatum can cooperate with other intestinal microorganisms to regulate the tumor immune microenvironment and promote the progression of colorectal cancer, and its high abundance is often associated with poor prognosis and drug resistance in the late stage of chemotherapy[13]. Therefore, the exploration of molecular targets targeting F. nucleatum to inhibit the progression of colorectal cancer is reliable. This part summarizes the existing potential therapeutic targets and molecular mechanisms related to F. nucleatum.
Pathogenic mechanism
FadA is a F. nucleatum adhesin that can bind to E-cadherin on human colon epithelial cells, activating the Wnt/β-catenin signaling pathway. This promotes CRC cell proliferation, migration, and invasion[14]. Fn infection can also prompt CRC development by stimulating toll-like receptor 4 (TLR4) signaling, resulting in NF-κB activation and upregulation of the pro-carcinogenic microRNA-21 (miR-21)[15]. Lipopolysaccharide (LPS), a cell wall component of F. nucleatum, can bind to TLR4 on the surface of tumor cells, activating MyD88 and other signaling pathways. This will further promote the transformation of LC3-I to LC3-II and the formation of autophagosomes, contributing to the degradation of chemotherapy drugs and resistance. Activation of the TLR4-MyD88 signaling pathway can also inhibit the expression of two microRNAs involved in autophagy signaling molecules, thereby further enhancing the activation of autophagy[16].
Therapeutic targets
Current approaches to impede F. nucleatum-mediated CRC progression primarily fall into three categories:
(1) Small molecule compound inhibitors. Previous studies have shown that CEACAM1 and TIGIT inhibitors can be used as potential adjuvant drugs for CRC[17]. At present, some small molecule drugs under clinical trial are screened, such as azelnidipine and liothyronine[18]. A recent study has shown that Sodium New Houttuyfonate (SNH) significantly inhibits the progression of CRC by targeting F. nucleatum's membrane-associated protein FadA, leading to FadA polymerization, membrane rupture, and penetration, which blocks the tumor-promoting activity of F. nucleatum and F. nucleatum-associated cancer-driven inflammation, protecting the intestinal barrier[19]. Studies have also shown that antimicrobial peptides (AMPs) are a new class of antimicrobial drugs. Br-J-I, a derivative of antimicrobial peptides Jelleine-I, inhibits F. nucleatum colonization, colon inflammation, and F. nucleatum-induced CRC growth by targeting FadA. Br-J-I can also enhance the antitumor effect of 5-fluorouracil[20].
(2) Phage-guided intestinal microbiome regulation therapy. Irinotecan-loaded dextran nanoparticles covalently linked to azide-modified phages were demonstrated to selectively inhibit the proliferation of F. nucleatum and autophagy of colon tumor, thus restoring the sensitivity of tumor cells to chemotherapy drugs[21].
Bacteroides fragilis
Bacteroides fragilis is divided into nontoxigenic Bacteroides fragilis (NTBF) and enterotoxigenic Bacteroides fragilis (ETBF) according to the ability to produce Bacteroides fragilis toxin (BFT). ETBF is an obligate anaerobic gram-negative bacterium that can secrete BFT to destroy the intestinal epithelial barrier, induce inflammation and precancerous lesions, and ultimately promote the tumorigenesis and progression of colorectal cancer[22].
Pathogenic mechanism
In a steady state, mucosal barrier can separate gut microbiota from the immune system. Destruction of mucosal barrier will cause chronic inflammation, which will further inhibit apoptosis of abnormal cells and eventually lead to cancer. Wu et al. found that Bacteroides fragilis toxin (BFT) produced by ETBF destroys colon mucosal barrier by degrading E-cadherin of colon epithelial cells[23]. BFT could also induce Wnt/β-catenin signaling[24]. In addition, the transcription factor STAT3 in colon epithelial cells and immune cells activated by ETBF could induce the production of growth factors, promote survival and proliferation of tumor cells, and inhibit antitumor immune response[25]. ETBF was also involved in the cytokine secretion of T cells, which would further increase the risk of intestinal inflammation and cancer[26]. ETBF can also affect the inflammatory response of cells, the degradation of extracellular matrix, and the regulation of gene expression through various signaling pathways, such as NF-κB and MAPK signaling pathways, which will further promote tumorigenesis and cancer progression[27,28]. Biofilms are structures composed of bacteria and extracellular polymers that protect bacteria from being attacked by the host immune system and antimicrobial agents[29]. ETBF could participate in biofilm formation and alter the tumor microenvironment, thereby promoting carcinogenesis[30].
Therapeutic targets
Currently, the potential anti-enterotoxigenic Bacteroides fragilis therapy to inhibit CRC progression can be divided into three categories:
(1) Natural product screening. Kim et al. found that zerumbone could effectively inhibit biofilm formation in Bacteroides fragilis containing toxic BFT-2 by downregulating the effector pump gene (bmeB12) associated with biofilm formation[29]. α-humulene is also effective in killing enterotoxigenic Bacteroides fragilis and destroying biofilms, which is expected to treat intestinal infections[31]. In addition, a recent study showed that natural polysaccharides extracted from agricultural wastes exhibited antibacterial effects through involvement in DNA damage and plasma membrane permeability alteration in carcinogenic bacteria[32].
(2) Anti-inflammatory drugs. Administration of the anti-inflammatory drug auranofin can inhibit bacterial survival and eradicate bacterial biofilms, alleviating intestinal inflammatory response caused by ETBF, and may reduce the occurrence of inflammation-related cancers by reducing the expression of outer membrane protein (OmpA) gene and effector pump-related gene bmeB3[33].
(3) Probiotic therapy. The use of probiotic blends such as Clostridium butyricum and Bifidobacterium can regulate the intestinal microecological balance by inhibiting the production of intestinal pathogenic biofilms, thereby inhibiting the colonization and proliferation of ETBF, and reducing the possibility of intestinal inflammation and cancer[34]. It was also shown in the article that the synthetic recombinant BFT-2 can inhibit the incidence of colon cancer, by decreasing the expression of Ki-67 and increasing the expression of Caspase-3, leading to apoptosis of colon cancer cells[35].
Clostridium butyricum
Clostridium butyricum (CB) is a spore-bearing, gram-positive anaerobic bacterium of the genus Clostridium. It can inhibit the growth of intestinal pathogenic bacteria and promote the amplification of intestinal probiotics such as Bifidobacterium. In addition, Clostridium butyricum ferments undigested dietary fiber to produce short-chain fatty acids (SCFA), by which butyric acid is one of the main products. These SCFAs affect host intestinal homeostasis and barrier function.
Therapeutic targets
Clostridium butyricum can enhance the mucosal barrier by increasing MUC gene expression in goblet cells and thereby increasing the content of mucin[36]. Zhou et al. found that CB inhibited the occurrence and progression of colon cancer by regulating the levels of inflammatory factors such as IL-6 and IL-10 through the MyD88-NF-κB signaling pathway[37]. Another study showed that CB can reduce the proliferation and promote apoptosis of intestinal tumor cells by inhibiting the Wnt/β-catenin signaling pathway. It can also reduce the secondary bile acid content in the stool, and increase the SCFA content in the cecum, which could further activate G protein-coupled receptors (GPRs) and activate the antiproliferative effect of probiotics[38]. Xu et al. found that Clostridium butyricum can also reduce the stability of MYC protein by enhancing ubiquitin-mediated degradation, which contributes to the inhibition of the MYC-TYMS signaling pathway involved in CRC[39]. In addition, Clostridium butyricum can also inhibit the progression of colorectal cancer by regulating the expression levels of TGF-β, miR-200c, and Bcl-2[40,41].
Previous studies have reported Prebiotics-Encapsulated Probiotic Spores, spores-dex, which could be specifically enriched in colon cancer lesions after oral administration. Oligosaccharides are fermented by Clostridium butyricum to produce anticancer short-chain fatty acids (SCFAs). Meanwhile, spores-dex could also regulate the gut microbiota, increase the abundance of SCFA-producing bacteria, and significantly increase the overall diversity of the microbiota[42].
Streptococcus gallolyticus
Streptococcus gallolyticus, formerly known as Streptococcus bovis, is a Gram-positive pathogenic bacteria that causes endocarditis in humans. It is also the first bacterium identified to be associated with the development of CRC[43,44]. Studies have shown that patients with Streptococcus gallolyticus infection have an elevated risk of developing CRC[44]. In 2021, Rahwa Taddese et al. found that S. gallolyticus interrelates with AhR-mediated pathways for cellular biotransformation (CYP1), which could potentially contribute to combating DNA damage and/or play a role in removing intestinal pathogens and protecting the epithelial barrier. S. gallolyticus increased the expression and activity of CYP1 biotransformation capacity in colorectal epithelial cells[45].
Peptostreptococcus anaerobius
Peptostreptococcus anaerobius is an anaerobic bacterium selectively enriched in the fecal and mucosal microbiota of CRC patients[46]. In 2019, Long et al. found that, in vivo, anaerobic bacteria attach to the mucosa of colorectal cancer, accelerating the development of colorectal cancer; anaerobes selectively adhere to colorectal cancer cell lines in vitro. Mechanistically, anaerobic bacteria drive colorectal cancer through the PCWBR2-integrin α2/β1-PI3K-AKT-Nf-κb signaling axis, and the PCWBR2-integrin α2/β1 axis is identified as a potential therapeutic target for CRC. α2/β1 is a receptor frequently overexpressed in human CRC tumors and cell lines. PCWBR2 can interact with α2/β1, which can induce the activation of the PI3K–Akt pathway in CRC cells. This leads to increased cell proliferation and NF-κB activation[47].
The pathogenic microbiota and their therapeutic targets are summarized in Table 1.
Gut microbiota in CRC-targeted therapy
Bacteria | Animal model&Cell line | Targeting sites/pathways | Therapies and drugs |
Fusobacterium nucleatum (F. nucleatum) | BALB/c nude mice (HCT116); AOM/DSS; HCT116, DLD1, SW480, HT29, LoVo, SW480, CT26 | Activating Wnt/β-catenin signaling pathway[14]; Activating NF-κB signaling pathway and upregulating miR-21[15]; Activating MyD88 signaling pathway[16] | Small molecule compound inhibitors: Azelnidipine and liothyronine[18]. Sodium New Houttuyfonate (SNH)[18]. Antimicrobial peptides (AMPs)[20]. Phage-guided therapy[21] |
Bacteroides fragilis | ApcMin/+ mice | E-cadherin of colon epithelial cells[23]. Activating STAT3[25]. Activating NF-κB signaling pathway[27] | Natural product : zerumbone, α-humulene, polysaccharides[29] Anti-inflammatory drugs: auranofin[33]. Probiotic therapy[35] |
Clostridium butyricum (CB) | BALB/c nude mice (HCT116); AOM/DSS; HCT116, DLD1, RKO | Inhibiting MyD88-NF-κB signal pathway[37]; Inhibiting Wnt/β-catenin signaling pathway[38]; Inhibiting MYC-TYMS signaling pathway[39] | 1. Increase the SCFA content and activate probiotics[38]. 2. Prebiotics-Encapsulated Probiotic Spores[42] |
Streptococcus gallolyticus (S.gallolyticus) | HT-29, SW480, HCT116, Caco-2 | Inducing CYP1[45] | / |
Peptostreptococcus anaerobius | ApcMin/+ mice, HT-29, Caco-2, NCM460 | Activating α2/β1-PI3K-Akt-NF-κB signalling axis[47] | Potential therapeutic targets:PCWBR2-integrin α2/β1 axis[47] |
MICROBIOTA IN IMMUNOTHERAPY
Gram negative bacillus
Gram-negative bacillus generally refers to bacteria with red Gram staining reaction. It mainly includes plateau Escherichia coli and Pseudomonas aeruginosa. LPS is one of the important products of Gram-negative bacteria. As an immunostimulatory ligand, LPS can activate TLR4 and nuclear factor NF-κB pathway. As early as 2011, Hsu et al. found that LPS could promote CRC liver metastasis by stimulating the TLR4 signaling pathway and increasing cell adhesion mediated by β1 integration[48]. In 2018, Song et al. found that LPS content was significantly increased in the blood and tissues of patients with early adenoma and CRC. High levels of LPS in orthotopic colon cancer tissues were associated with low response to PD-L1 monoclonal antibody treatment in immunotherapy. Clearance of Gram-negative bacteria in gut with polymyxin B (PmB) or blockade of TLR4 with TAK-242 alleviated the immunosuppressive microenvironment and promoted T-cell infiltration into CRC tumors. Based on this finding, Song et al. considered neutralizing or modulating LPS produced by gut microbes as a potential treatment for colorectal cancer. Therefore, they suggest that genetically engineered bacteria that secrete LPS traps or PmB produced by Bacillus polymyxa are promising therapeutic approaches to kill Gram-negative bacillus instead of antibiotics. Transplanting these bacteria into the host gut in an appropriate manner could be an effective trial to clear LPS and regulate the CRC microenvironment[49].
Lachnospiraceae
Lachnospiraceae belongs to Firmicutes, an obligate anaerobes found in most healthy people. Lachnospiraceae is involved in the production of SCFAs, an important energy source for intestinal epithelial cells[50]. Spirochetes participate in host immune system by producing short-chain fatty acids, converting primary to secondary bile acids, and promoting colonization resistance to enteric pathogens[51]. In 2023, Zhang et al. found that commensal bacteria Ruminococcus (Rg), Bacillus (Bp), and Proteus (Df) of the Spirillaceae family were enriched in normal tissues, while Fusobacterium nucleatum (Fn) and anaerobic Streptococcus gasteri (Pa) were enriched in tumor tissues. Depletion of Rg and Bp promoted the antitumor effect of CD8+ T cells, and thus inhibited the progression of CRC, which could be a potential combination approach with immunotherapy[52].
Lactobacillus gallinarum
In 2022, Sugimura et al. identified Lactobacillus gallinarum as a potential adjuvant that could enhance the effect of anti-PD1 immunotherapy on CRC. Indole-3-lactic acid (ILA), a tryptophan metabolite produced by Lactobacillus gallinarum, can be further derived to indole-3-carboxylic acid (ICA), a functional metabolite that could inhibit the production of kynurenine (Kyn) in tumors by inhibiting the expression of indoleamine 2,3-dioxygenase (IDO1). In addition, Lactobacillus gallinarum-derived ICA competed with Kyn for the binding site of AHR and antagonized the binding of Kyn to CD4+ T cells. In conclusion, Lactobacillus gallinarum enhanced the efficiency of anti-PD1 therapy in CRC by inhibiting differentiation of Treg, and enhancing CD8+ T cell function through IDO1/Kyn/AHR axis[53].
Streptococcus thermophilus
Streptococcus thermophilus is a Gram-positive anaerobes that protects the intestinal epithelium by preventing invasive Escherichia coli[54]; it has also been shown to increase ceramide levels both in vitro and in vivo[55]. Li et al. found in 2021 that S. thermophilus inhibited colorectal tumorigenesis by secreting β-galactosidase. β-galactosidase secreted by S. thermophilus inhibits cell proliferation, reduces colony formation, induces cell cycle arrest, promotes CRC cell apoptosis, and retards the growth of CRC xenografts. Mutant S. thermophilus without functional β-galactosidase loses its tumor suppressive effect. As a novel drug for CRC prevention, S. thermophilus has a promising application prospect[56].
Lactobacillus paracasei
Lactobacillus paracasei is a Gram-positive and facultative anaerobic fermentative Lactobacillus that is widely distributed in fermented dairy products, vegetables, cereal products, and the gastrointestinal tract of humans and animals[57]. In recent years, L. paracasei has been shown to have a variety of health effects, such as antitumor immunity, improvement of functional dyspepsia, and improvement of lipid metabolism[58,59]. In 2018, Chang et al. found that the combination of NTU101-FM extract with 5-fluorouracil (5-FU), a chemotherapeutic drug, could induce CRC cell viability without toxicity to colonic epithelial cells[60]. In 2022, Zhang et al. demonstrated that L. paracasei sh2020 could induce antitumor immunity in mice and synergize with anti-PD-1 to delay tumor progression. The team found that the microbiome from healthy individuals had considerable sensitivity to anti-PD-1, which was absent from the gut microbiome of CRC patients. L. paracasei sh2020 was identified to exhibit significant antitumor immunity in mice with gut dysbiosis. Mechanistically, L. paracasei sh2020 stimulation led to the upregulation of CXCL10 expression in tumors and enhanced CD8+ T cell recruitment[57]. In the same year, Shi et al. found that L. paracasei PC-H1 could use extracellular vesicles to induce apoptosis of colorectal cancer cells and delay the progression of CRC by using the PDK1/AKT/Bcl-2 signaling pathway[61]. Taken together, the regulation of intestinal microbiota by L. paracasei may play a synergistic role in enhancing immunotherapy and provide a new target for the treatment of CRC, which may have a broad prospect in the clinical treatment of CRC.
Clostridium
Clostridium is a general term for gram-positive, anaerobic, microaerobic and coarse bacillus species. Montalban et al. found that the abundance of Clostridium was decreased in CRC patients. Mice administrated orally with four Clostridium species (CC4) exhibited the activation of IFN-γ+, granzyme B+, CD8+ T cells and downregulation of immune checkpoint molecules, which resulted in the activation of antitumor immune response and further CRC inhibition[62].
Fusobacterium nucleatum
In addition to targeted treatment of CRC, Fusobacterium nucleatum can also work with immune pathways to inhibit CRC progression. TIGIT (T cell Ig and ITIM domain) is an inhibitory receptor expressed on regulatory T cells (Treg), activated T cells, and natural killer cells (NK cells). CEACAM1 is a cell adhesion molecule involved in tumor metastasis by binding to TIGIT. Fap2 is an outer membrane protein capable of binding to TIGIT/CEACAM1, thereby inhibiting the activity of natural killer cells and promoting the development and progression of CRC[63]. Specific antibodies targeting anti-FAP2 protein antibodies/anti-CEACAM1 and TIGIT can be used to block the interaction between Fn and immune cells, thereby enhancing the function of natural killer cells and inhibiting the progression of colorectal cancer.
We summarized the mechanism of action and target cells of gut microbiota in CRC- immunotherapy in Table 2.
Gut microbiota in CRC- immunotherapy
Bacteria | Animal model&cell line | Targeted cell | Mechanism |
Lachnospiraceae | ApcMin/+ mice; MC38, CT26 | CD8+ T cell | Promoting the antitumor effect of CD8+ T cell[47] |
Lactobacillus gallinarum | AOM/DSS; HCT116, LoVo, MC38 | CD4+ T cell CD8+ T cell | Inhibition of IDO1 expression, suppressing Kyn production; Inhibition of Treg differentiation[53] |
Lacticaseibacillus paracasei (L. paracasei) | MC38. | CD8+ T cell | Enhancing CD8+ T cell recruitment[57] |
Clostridium | AOM/DSS; MC38, CT26 | CD8+ T cell | Activation of CD8+ T cells[62] |
MICROBIOTA IN RELATION TO DIETS AND CRC
Dietary impacts and drug resistance
There are many other factors that influence the gut microbiota in CRC, and the current approach to analyzing these factors involves the concept of Molecular pathological epidemiology. Molecular pathological epidemiology (MPE) is based on molecular pathology and disease heterogeneity, using epidemiological study design methods to comprehensively analyze the effects of exposure factors, lifestyle habits, and changes at the molecular level on disease development, prognosis, and outcomes[64,65]. Therefore, analyzing the impact of factors such as diet on disease progression in a clinical sample of colorectal cancer patients is a novel and interesting aspect.
Yang et al. found that a high-fat diet can lead to an increase in some bacteria associated with colorectal cancer in the intestinal tract of mice, and a high-fat diet can alter the levels of intestinal metabolites, for example, an increase in the expression of lysophosphatidic acid (LPA) can stimulate proliferation and inflammation of intestinal epithelial cells[66].
In addition, a high-fat diet can impair the function of the intestinal epithelial barrier, making the gut more vulnerable to bacteria and metabolites. It has also been shown that high-fat diets and APC mutations can alter bile acid (BA) levels in the body, leading to proliferation and DNA damage in intestinal stem cells, which promotes the formation and progression of CRC[67].
In addition, calorie restriction, fasting food during fasting phase, Vitamin D and ketone bodies can decrease CRC risk in humans/animal models while fructose in diets can increase it in animal models and some epidemiological associations[68]. All of the above evidence suggests a correlation between diet and the development of colorectal cancer, and diet control may be able to serve as a new target and strategy for the prevention and treatment of colorectal cancer.
The emergence of drug-resistant pathogens can also have an impact on the efficacy of clinical treatment. The presence of antibiotic resistance genes (ARGs) in the microbiota is characterized by quantity, identity, and function, which are collectively referred to as resistome[69]. There are already a number of strategies to combat drug-resistant microorganisms, such as those that utilize the CRISPR Cas9 system to eliminate selected bacterial targets[70]. Furthermore, combining molecular pathological epidemiology with microbial resistance and other factors, the strategy of rationally creating treatment methods to achieve better clinical treatment outcomes may have broad prospects.
Probiotic interventions
Studies have shown that the composition of gut microbiota changes significantly with the progression of CRC[71]. Driver bacteria increase in the early stages of CRC, providing conditions for tumor formation. Passenger bacteria are able to maintain CRC through metabolites. Furthermore, some probiotics can inhibit the progression of colorectal cancer. In conclusion, alterations in the ratio of gut microbiota may lead to dysregulation of homeostasis in the intestinal environment. Therefore, effective utilization of gut microbiota that play different functions in CRC may provide new ideas and methods for its prevention and treatment.
Notable among these is probiotic supplementation. Probiotics are active bacteria isolated from the gut or fermented foods that can be used as food or food supplements for improving gut microbiota homeostasis or for preventive treatment of specific diseases[72]. Some studies have shown that patients who underwent surgical resection of CRC displayed a decrease in CRC-associated bacteria (Fusobacterium, Porphyromonas, and Alloprevotella) after being given probiotic (Clostridium butyricum, Lactobacillus plantarum, and Bifidobacterium) treatments and a reduction in postoperative complications[73,74].
CONCLUSION
Although our current understanding of microorganisms is limited, it is undeniable that microorganisms, as an important part of the animal body, have broad therapeutic prospects. In recent years, more and more attention has been paid to the study of gut microbes. Existing studies confirmed that the efficiency of gut microbiota has been extensively studied in CRC treatment, and certain results have been achieved, such as the enhancement of activation of CD8+ T cells by microorganisms and the enhancement of anti-PD-1 immunotherapy. This signals that gut microbes may be able to act as an adjuvant to enhance immunotherapy. In addition, intestinal microbes can also use some targeted pathways, such as the MyD88-NF-κB signaling pathway, to regulate the levels of inflammatory factors such as IL-6 and IL-10, thereby inhibiting CRC progression. However, most of the results have only been verified in vivo in mice and have not been applied in clinical practice. Due to the similarities in the immune environment between humans and mice, there are still great limitations in clinical application.
Despite many difficulties, current research still shows that therapeutic strategies targeting microbiota will become a key area of personalized cancer treatment, and the strategies of microbes as adjuvants in various therapies also have broad prospects.
DECLARATIONS
Authors’ contributions
Made substantial contributions to the conception and design of the study and performed data analysis and interpretation: Zhou X, Zhao Y, Zhao R, Shafi S, Yang Y
Performed data acquisition, as well as providing administrative, technical, and material support: Liu SB, Liu G
Availability of data and materials
Not applicable.
Financial support and sponsorship
This study was supported by the Project of Jiangsu Province Engineering Research Center of Molecular Target Therapy and Companion Diagnostics in Oncology, Jiangsu higher education institution innovative research team for science and technology (2021), Program of Jiangsu vocational college engineering technology research center (2023), Key technology program of Suzhou people's livelihood technology projects (Grant No. SKY2021029), Key Programs of the Suzhou Vocational Health College (SZWZYTD202201), Qing‐Lan Project of Jiangsu Province in China (2021, 2022).
Conflicts of interest
All authors declared that there are no conflicts of interest.
Ethical approval and consent to participate
Not applicable.
Consent for publication
Not applicable.
Copyright
© The Author(s) 2024.
REFERENCES
1. White MT, Sears CL. The microbial landscape of colorectal cancer. Nat Rev Microbiol 2023; doi: 10.1038/s41579-023-00973-4.
2. Rassy E, Parent P, Lefort F, Boussios S, Baciarello G, Pavlidis N. New rising entities in cancer of unknown primary: Is there a real therapeutic benefit? Crit Rev Oncol Hematol 2020;147:102882.
3. Ohishi T, Kaneko MK, Yoshida Y, Takashima A, Kato Y, Kawada M. Current targeted therapy for metastatic colorectal cancer. Int J Mol Sci 2023;24:1702.
4. Osseis M, Nehmeh WA, Rassy N, et al. Surgery for T4 colorectal cancer in older patients: determinants of outcomes. J Pers Med 2022;12:1534.
5. Leowattana W, Leowattana P, Leowattana T. Systemic treatment for metastatic colorectal cancer. World J Gastroenterol 2023;29:1569-88.
6. Augusti PR, Quatrin A, Mello R, et al. Antiproliferative effect of colonic fermented phenolic compounds from jaboticaba (myrciaria trunciflora) fruit peel in a 3D cell model of colorectal cancer. Molecules 2021;26:4469.
7. Adeleke S, Haslam A, Choy A, et al. Microsatellite instability testing in colorectal patients with Lynch syndrome: lessons learned from a case report and how to avoid such pitfalls. Per Med 2022;19:277-86.
8. Ganesh K, Stadler ZK, Cercek A, et al. Immunotherapy in colorectal cancer: rationale, challenges and potential. Nat Rev Gastroenterol Hepatol 2019;16:361-75.
9. Chen M, Lin W, Li N, et al. Therapeutic approaches to colorectal cancer via strategies based on modulation of gut microbiota. Front Microbiol 2022;13:945533.
10. Cheng Y, Ling Z, Li L. The intestinal microbiota and colorectal cancer. Front Immunol 2020;11:615056.
11. Alon-Maimon T, Mandelboim O, Bachrach G. Fusobacterium nucleatum and cancer. Periodontol 2000 2022;89:166-80.
12. Brennan CA, Garrett WS. Fusobacterium nucleatum - symbiont, opportunist and oncobacterium. Nat Rev Microbiol 2019;17:156-66.
13. Kostic AD, Chun E, Robertson L, et al. Fusobacterium nucleatum potentiates intestinal tumorigenesis and modulates the tumor-immune microenvironment. Cell Host Microbe 2013;14:207-15.
14. Rubinstein MR, Wang X, Liu W, Hao Y, Cai G, Han YW. Fusobacterium nucleatum promotes colorectal carcinogenesis by modulating E-cadherin/β-catenin signaling via its FadA adhesin. Cell Host Microbe 2013;14:195-206.
15. Yang Y, Weng W, Peng J, et al. Fusobacterium nucleatum increases proliferation of colorectal cancer cells and tumor development in mice by activating toll-like receptor 4 signaling to nuclear factor-κB, and up-regulating expression of microRNA-21. Gastroenterology 2017;152:851-866.e24.
16. Park BS, Lee JO. Recognition of lipopolysaccharide pattern by TLR4 complexes. Exp Mol Med 2013;45:e66.
17. Zhou X, Jiao L, Qian Y, et al. Repositioning azelnidipine as a dual inhibitor targeting CD47/SIRPα and TIGIT/PVR pathways for cancer immuno-therapy. Biomolecules 2021;11:706.
18. Thuru X, Magnez R, El-Bouazzati H, Vergoten G, Quesnel B, Bailly C. Drug repurposing to enhance antitumor response to PD-1/PD-L1 immune checkpoint inhibitors. Cancers (Basel) 2022;14:3368.
19. Jia F, Yu Q, Zhao L, Shen Y, Guo H, He F. Sodium new houttuyfonate inhibits cancer-promoting fusobacterium nucleatum (Fn) to reduce colorectal cancer progression. Cancers (Basel) 2022;14:6111.
20. Jia F, Yu Q, Wang R, et al. Optimized antimicrobial peptide jelleine-I derivative Br-J-I inhibits fusobacterium nucleatum to suppress colorectal cancer progression. Int J Mol Sci 2023;24:1469.
21. Zheng DW, Dong X, Pan P, et al. Phage-guided modulation of the gut microbiota of mouse models of colorectal cancer augments their responses to chemotherapy. Nat Biomed Eng 2019;3:717-28.
22. Sun F, Zhang Q, Zhao J, Zhang H, Zhai Q, Chen W. A potential species of next-generation probiotics? Food Res Int 2019;126:108590.
23. Wu S, Rhee KJ, Zhang M, Franco A, Sears CL. Bacteroides fragilis toxin stimulates intestinal epithelial cell shedding and gamma-secretase-dependent E-cadherin cleavage. J Cell Sci 2007;120:1944-52.
25. Wang L, Yi T, Zhang W, Pardoll DM, Yu H. IL-17 enhances tumor development in carcinogen-induced skin cancer. Cancer Res 2010;70:10112-20.
26. Brockmann L, Giannou AD, Gagliani N, Huber S. Regulation of T(H)17 cells and associated cytokines in wound healing, tissue regeneration, and carcinogenesis. Int J Mol Sci 2017;18:1033.
27. Shaked H, Hofseth LJ, Chumanevich A, et al. Chronic epithelial NF-κB activation accelerates APC loss and intestinal tumor initiation through iNOS up-regulation. Proc Natl Acad Sci U S A 2012;109:14007-12.
28. Kwon MJ, Hong E, Choi Y, Kang DH, Oh ES. Interleukin-1α promotes extracellular shedding of syndecan-2 via induction of matrix metalloproteinase-7 expression. Biochem Biophys Res Commun 2014;446:487-92.
29. Kim HR, Rhee KJ, Eom YB. Anti-biofilm and antimicrobial effects of zerumbone against Bacteroides fragilis. Anaerobe 2019;57:99-106.
30. Li S, Konstantinov SR, Smits R, Peppelenbosch MP. Bacterial biofilms in colorectal cancer initiation and progression. Trends Mol Med 2017;23:18-30.
31. Jang HI, Rhee KJ, Eom YB. Antibacterial and antibiofilm effects of α-humulene against Bacteroides fragilis. Can J Microbiol 2020;66:389-99.
32. Al-Hazmi NE, Naguib DM. Control the carcinogenic bacteria with new polysaccharides from agricultural wastes. Microb Pathog 2023;184:106343.
33. Jang HI, Eom YB. Antibiofilm and antibacterial activities of repurposing auranofin against Bacteroides fragilis. Arch Microbiol 2020;202:473-82.
34. Abdelhamid AG, Esaam A, Hazaa MM. Cell free preparations of probiotics exerted antibacterial and antibiofilm activities against multidrug resistant E. coli. Saudi Pharm J 2018;26:603-7.
35. Lv Y, Ye T, Wang HP, et al. Suppression of colorectal tumorigenesis by recombinant Bacteroides fragilis enterotoxin-2 in vivo. World J Gastroenterol 2017;23:603-13.
36. Liu M, Xie W, Wan X, Deng T. Clostridium butyricum modulates gut microbiota and reduces colitis associated colon cancer in mice. Int Immunopharmacol 2020;88:106862.
37. Zhou M, Yuan W, Yang B, Pei W, Ma J, Feng Q. Clostridium butyricum inhibits the progression of colorectal cancer and alleviates intestinal inflammation via the myeloid differentiation factor 88 (MyD88)-nuclear factor-kappa B (NF-κB) signaling pathway. Ann Transl Med 2022;10:478.
38. Chen D, Jin D, Huang S, et al. Clostridium butyricum, a butyrate-producing probiotic, inhibits intestinal tumor development through modulating Wnt signaling and gut microbiota. Cancer Lett 2020;469:456-67.
39. Xu H, Luo H, Zhang J, Li K, Lee MH. Therapeutic potential of Clostridium butyricum anticancer effects in colorectal cancer. Gut Microbes 2023;15:2186114.
40. Xin M, Xie Q, Ma L, et al. Synergistic anti-tumour effects of Clostridium butyricum in combination with apatinib in CT26 colorectal tumour-bearing mice. Anticancer Drugs 2019;30:991-7.
41. Xiao Y, Dai X, Li K, Gui G, Liu J, Yang H. Clostridium butyricum partially regulates the development of colitis-associated cancer through miR-200c. Cell Mol Biol (Noisy-le-grand) 2017;63:59-66.
42. Zheng DW, Li RQ, An JX, et al. Prebiotics-encapsulated probiotic spores regulate gut microbiota and suppress colon cancer. Adv Mater 2020;32:e2004529.
43. Boleij A, Tjalsma H. The itinerary of Streptococcus gallolyticus infection in patients with colonic malignant disease. Lancet Infect Dis 2013;13:719-24.
44. Kumar R, Herold JL, Schady D, et al. Streptococcus gallolyticus subsp. gallolyticus promotes colorectal tumor development. PLoS Pathog 2017;13:e1006440.
45. Taddese R, Roelofs R, Draper D, et al. Streptococcus gallolyticus increases expression and activity of aryl hydrocarbon receptor-dependent CYP1 biotransformation capacity in colorectal epithelial cells. Front Cell Infect Microbiol 2021;11:740704.
46. Tsoi H, Chu ESH, Zhang X, et al. Peptostreptococcus anaerobius induces intracellular cholesterol biosynthesis in colon cells to induce proliferation and causes dysplasia in mice. Gastroenterology 2017;152:1419-1433.e5.
47. Long X, Wong CC, Tong L, et al. Peptostreptococcus anaerobius promotes colorectal carcinogenesis and modulates tumour immunity. Nat Microbiol 2019;4:2319-30.
48. Hsu RY, Chan CH, Spicer JD, et al. LPS-induced TLR4 signaling in human colorectal cancer cells increases beta1 integrin-mediated cell adhesion and liver metastasis. Cancer Res 2011;71:1989-98.
49. Song W, Tiruthani K, Wang Y, et al. Trapping of lipopolysaccharide to promote immunotherapy against colorectal cancer and attenuate liver metastasis. Adv Mater 2018;30:e1805007.
50. Zhuang P, Zhang Y, Shou Q, et al. Eicosapentaenoic and Docosahexaenoic Acids differentially alter gut microbiome and reverse high-fat diet-induced insulin resistance. Mol Nutr Food Res 2020;64:e1900946.
51. Sorbara MT, Littmann ER, Fontana E, et al. Functional and genomic variation between human-derived isolates of lachnospiraceae reveals inter- and intra-species diversity. Cell Host Microbe 2020;28:134-146.e4.
52. Zhang X, Yu D, Wu D, et al. Tissue-resident Lachnospiraceae family bacteria protect against colorectal carcinogenesis by promoting tumor immune surveillance. Cell Host Microbe 2023;31:418-432.e8.
53. Sugimura N, Li Q, Chu ESH, et al. Lactobacillus gallinarum modulates the gut microbiota and produces anti-cancer metabolites to protect against colorectal tumourigenesis. Gut 2021;71:2011-21.
54. Hill ID, Dirks MH, Liptak GS, et al. North American Society for Pediatric Gastroenterology; Hepatology and Nutrition. Guideline for the diagnosis and treatment of celiac disease in children: recommendations of the North American Society for Pediatric Gastroenterology, Hepatology and Nutrition. J Pediatr Gastroenterol Nutr 2005;40:1-19.
55. Marzio L, Cinque B, De Simone C, Cifone MG. Effect of the lactic acid bacterium Streptococcus thermophilus on ceramide levels in human keratinocytes in vitro and stratum corneum in vivo. J Invest Dermatol 1999;113:98-106.
56. Li Q, Hu W, Liu WX, et al. Streptococcus thermophilus Inhibits colorectal tumorigenesis through secreting β-galactosidase. Gastroenterology 2021;160:1179-1193.e14.
57. Zhang SL, Han B, Mao YQ, et al. Lacticaseibacillus paracasei sh2020 induced antitumor immunity and synergized with anti-programmed cell death 1 to reduce tumor burden in mice. Gut Microbes 2022;14:2046246.
58. Sun E, Zhang X, Zhao Y, et al. Beverages containing Lactobacillus paracasei LC-37 improved functional dyspepsia through regulation of the intestinal microbiota and their metabolites. J Dairy Sci 2021;104:6389-98.
59. Lv XC, Chen M, Huang ZR, et al. Potential mechanisms underlying the ameliorative effect of Lactobacillus paracasei FZU103 on the lipid metabolism in hyperlipidemic mice fed a high-fat diet. Food Res Int 2021;139:109956.
60. Chang CY, Pan TM. Anticancer and antimigration effects of a combinatorial treatment of 5-fluorouracil and lactobacillus paracasei subsp. paracasei NTU 101 fermented skim milk extracts on colorectal cancer cells. J Agric Food Chem 2018;66:5549-55.
61. Shi Y, Meng L, Zhang C, Zhang F, Fang Y. Extracellular vesicles of Lacticaseibacillus paracasei PC-H1 induce colorectal cancer cells apoptosis via PDK1/AKT/Bcl-2 signaling pathway. Microbiol Res 2021;255:126921.
62. Montalban-Arques A, Katkeviciute E, Busenhart P, et al. Commensal Clostridiales strains mediate effective anti-cancer immune response against solid tumors. Cell Host Microbe 2021;29:1573-1588.e7.
63. Gur C, Maalouf N, Shhadeh A, et al. Fusobacterium nucleatum supresses anti-tumor immunity by activating CEACAM1. Oncoimmunology 2019;8:e1581531.
64. Inamura K, Hamada T, Bullman S, Ugai T, Yachida S, Ogino S. Cancer as microenvironmental, systemic and environmental diseases: opportunity for transdisciplinary microbiomics science. Gut ;2022:2107-22.
65. Ogino S, Nowak JA, Hamada T, Milner DA Jr, Nishihara R. Insights into pathogenic interactions among environment, host, and tumor at the crossroads of molecular pathology and epidemiology. Annu Rev Pathol 2019;14:83-103.
66. Yang J, Wei H, Zhou Y, et al. High-fat diet promotes colorectal tumorigenesis through modulating gut microbiota and metabolites. Gastroenterology 2022;162:135-149.e2.
67. Fu T, Coulter S, Yoshihara E, et al. FXR regulates intestinal cancer stem cell proliferation. Cell 2019;176:1098-1112.e18.
68. Sedlak JC, Yilmaz ÖH, Roper J. Metabolism and colorectal cancer. Annu Rev Pathol 2023;18:467-92.
69. Schwartz DJ, Langdon AE, Dantas G. Understanding the impact of antibiotic perturbation on the human microbiome. Genome Med 2020;12:82.
70. Becattini S, Taur Y, Pamer EG. Antibiotic-induced changes in the intestinal microbiota and disease. Trends Mol Med 2016;22:458-78.
71. Kvakova M, Kamlarova A, Stofilova J, Benetinova V, Bertkova I. Probiotics and postbiotics in colorectal cancer: Prevention and complementary therapy. World J Gastroenterol 2022;28:3370-82.
72. Tripathy A, Dash J, Kancharla S, et al. Probiotics: a promising candidate for management of colorectal cancer. Cancers (Basel) 2021;13:3178.
73. Park IJ, Lee JH, Kye BH, et al. Effects of probiotics on the symptoms and surgical outcomes after anterior resection of colon cancer (POSTCARE): a randomized, double-blind, placebo-controlled trial. J Clin Med 2020;9:2181.
Cite This Article
Export citation file: BibTeX | RIS
OAE Style
Zhou X, Zhao Y, Zhao R, Shafi S, Yang Y, Liu G, Liu SB. Research progress of intestinal microbiota in targeted therapy and immunotherapy of colorectal cancer. J Cancer Metastasis Treat 2024;10:12. http://dx.doi.org/10.20517/2394-4722.2023.178
AMA Style
Zhou X, Zhao Y, Zhao R, Shafi S, Yang Y, Liu G, Liu SB. Research progress of intestinal microbiota in targeted therapy and immunotherapy of colorectal cancer. Journal of Cancer Metastasis and Treatment. 2024; 10: 12. http://dx.doi.org/10.20517/2394-4722.2023.178
Chicago/Turabian Style
Zhou, Xinying, Yu Zhao, Rongchuan Zhao, Shaheryar Shafi, Yue Yang, Guangxing Liu, Song-Bai Liu. 2024. "Research progress of intestinal microbiota in targeted therapy and immunotherapy of colorectal cancer" Journal of Cancer Metastasis and Treatment. 10: 12. http://dx.doi.org/10.20517/2394-4722.2023.178
ACS Style
Zhou, X.; Zhao Y.; Zhao R.; Shafi S.; Yang Y.; Liu G.; Liu S.B. Research progress of intestinal microbiota in targeted therapy and immunotherapy of colorectal cancer. J. Cancer. Metastasis. Treat. 2024, 10, 12. http://dx.doi.org/10.20517/2394-4722.2023.178
About This Article
Special Issue
Copyright
Data & Comments
Data
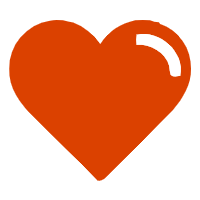

Comments
Comments must be written in English. Spam, offensive content, impersonation, and private information will not be permitted. If any comment is reported and identified as inappropriate content by OAE staff, the comment will be removed without notice. If you have any queries or need any help, please contact us at support@oaepublish.com.